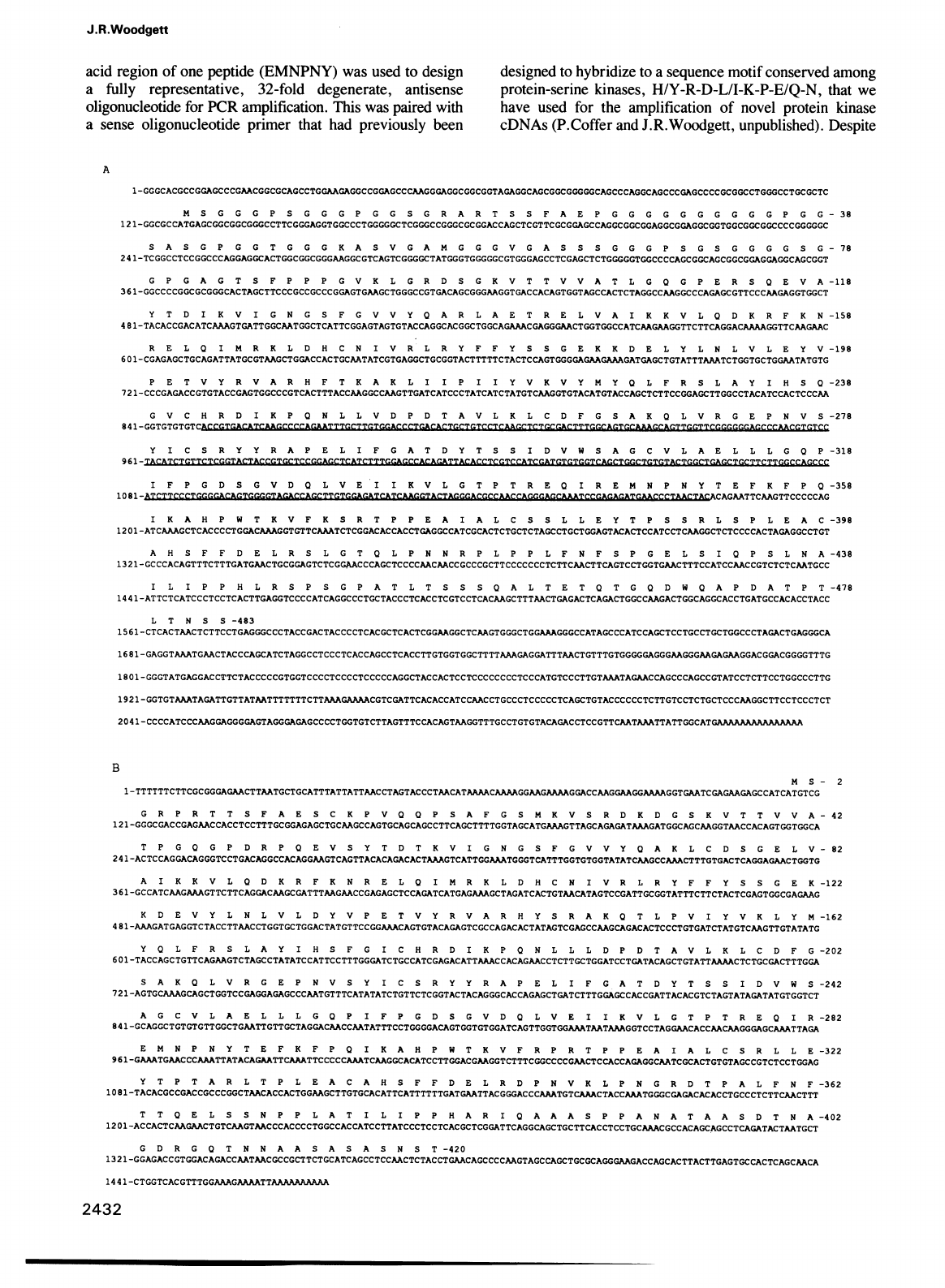
J.R.Woodgett
acid
region
of
one
peptide
(EMNPNY)
was
used
to
design
designed
to
hybridize
to
a
sequence
motif
conserved
among
a
fully
representative,
32-fold
degenerate,
antisense
protein-serine
kinases,
H/Y-R-D-L/I-K-P-E/Q-N,
that
we
oligonucleotide
for
PCR
amplification.
This
was
paired
with
have
used
for
the
amplification
of
novel
protein
kinase
a
sense
oligonucleotide
primer
that
had
previously
been
cDNAs
(P.Coffer
and
J.R.Woodgett,
unpublished).
Despite
A
1-
GGGCACGCCGGAGCCCGAACGGCGCAGCCTGGAAGAGGCCGGAGCCCAAGGGAGGCGGCGGTAGAGGCAGCGGCGGGGGCAGCCCAGGCAGCCCGAGCC
CCGCGGCCTGGGCCTGCGCTC
M
S
G
G
G
P S
G
G
G
P
G
G
S
G R
A
R
T
S
S
F
A
E
P
G
G G
G
G
G
G
G G
G
P
G
G
-
38
121-GGCGCCATGAGCGGCGGCGGGCCTTCGGGAGGTGGCCCTGGGGGCTCGGGCCGGGCGCGGACCAGCTCGTTCGCGGAGCCAGGCGGCGGAGGCGGAGGCGGTGGCGGCGGCCCCGGGGGC
S
A
S
G
P
G
G
T
G
G
G
K
A
S
V
G
A
M
G
G
G
V
G
A
S
S
S
G
G
G
P
S
G
S
G
G
G
G
S
G
-
78
241-TCGGCCTCCGGCCCAGGAGGCACTGGCGGCGGGAAGGCGTCAGTCGGGGCTATGGGTGGGGGCGTGGGAGCCTCGAGCTCTGGGGGTGGCCCCAGCGGCAGCGGCGGAGGAGGCAGCGGT
G
P
G
A
G
T
S
F
P
P P
G
V
K
L
G
R
D
S
G
K
V
T T
V
V
A
T
L
G
Q
G
P
E
R
S
0
E
V
A
-118
361-GGCCCCGGCGCGGGCACTAGCTTCCCGCCGCCCGGAGTGAAGCTGGGCCGTGACAGCGGGAAGGTGACCACAGTGGTAGCCACTCTAGGCCAAGGCCCAGAGCGTTCCCAAGAGGTGGCT
Y
T
D
I
K
V
I
G
N
G
S
F
G
V
V
Y
0
A
R
L
A
E T
R E
L
V
A
I
K
K
V
L
Q
D
K
R
F
K N
-158
4
81-
TACAC
CGACATCAAAGTGATTGGCAATGGCT
CATTCGGAGTAGTGTACCAGGCACGGCTGGCAGAAACGAGGGAACTGGTGGCCATCAAGAAGGTTCTTCAGGACAAAAGGTTCAAGAAC
R
E
L
0
I
M
R
K
L
D
H
C
N
I
V
R
L
R
Y
F
F
Y
S
S
G
E
K
K
D
E
L
Y
L
N
L
V
L
E
Y
V
-198
6
01-
CGAGAGC
TGCAGATTATGC
GTAAGCTGGACCAC
TGCAATATCGTGAGGCTGCGGTACTTTTTC
TACTCCAGTGGGGAGAAGAAAGATGAGCTGTATTTAAATCTGGTGCTGGAATATGTG
P
E
T
V
Y
R
V
A
R
H
F
T
K
A
K
L
I
I
P
I
I
Y
V
K
V
Y
M
Y
Q
L
F
R
S
L
A
Y
I
H
S
Q
-238
721-CCCGAGACCGTGTACCGAGTGGCCCGTCACTTTACCAAGGCCAAGTTGATCATCCCTATCATCTATGTCAAGGTGTACATGTACCAGCTCTTCCGGAGCTTGGCCTACATCCACTCCCAA
G
V
C
H
R
D
I
K
P
Q
N
L
L
V
D
P
D T
A
V
L
K
L
C
D F
G
S
A
K
Q
L
V
R
G
E
P
N
V
S
-278
841
-GGTGTGTGTC
Y
I
C
S
R Y
Y R
A
P
E
L
I
F G
A
T
D
Y
T
S
S
I
D
V
W
S
A
G
C
V
L
A
E
L
L
L
G
Q
P
-318
961-TACATCTGTTCTCGGTACT
GCTCCGG
CTCATCTTTGGAGCCACAGATTACACCTCGTCCATCGATGT
TGGTCAGC
GGCTGTGT
GCTGCTTCTTGGCCAGCC
I
F
P
G D
S
G
V
D
0
L
V
E
I
I
K
V
L
G
T
P
T
R
E
Q
I
R
E
M
N
P
N
Y
T
E
F
K
F
P
Q
-358
1081-ATCTTCCC
GGGACAGTGGGGTAGACCAGCTTGTGGAGATCATCAAGGTACTAGGr.ACr-CCAACCAGGGAGCAAA
GAACCCTAACTACACAGAATTCAAGTTCCCCCAG
I
K
A
H
P
W
T
K
V
F
K
S
R
T
P
P
E
A
I
A
L
C
S
S
L
L
E
Y
T
P
S
S
R
L
S P
L
E
A
C
-398
1201-ATCAAAGCTCACCCCTGGACAAAGGTGTTCAAATCTCGGACACCACCTGAGGCCATCGCACTCTGCTCTAGCCTGCTGGAGTACACTCCATCCTCAAGGCTCTCCCCACTAGAGGCCTGT
A
H
S
F
F
D
E
L
R
S
L
G
T
Q
L
P
N
N R
P
L
P
P
L
F
N
F
S
P
G
E
L
S
I
Q
P
S
L
N
A
-438
1321-GCCCACAGTTTCTTTGATGAACTGCGGAGTCTCGGAACCCAGCTCCCCAACAACCGCCCGCTTCCCCCCCTCTTCAACTTCAGTCCTGGTGAACTTTCCATCCAACCGTCTCTCAATGCC
I
L
I
P
P
H
L
R
S
P
S
G
P
A
T
L
T
S
S
S
Q
A
L
T
E
T
Q
T
G
Q
D
W
Q
A
P
D
A
T
P
T
-478
1441-ATTCTCATCCCTCCTCACTTGAGGTCCCCATCAGGCCCTGCTACCCTCACCTCGTCCTCACAAGCTTTAACTGAGACTCAGACTGGCCAAGACTGGCAGGCACCTGATGCCACACCTACC
L
T N
S
S
-483
15
61-
CT
CACTAACTCTTCCT
GAGGGC
CCTACCGACTACCC
CTCACGCTCACTCGGAAGGCTCAAGTGGGCTGGAAAGGGCCATAGCCCATCCAGCTCCTGCCTGCTGGCCCTAGACTGAGGGCA
16
81-
GAGGTAAATGAACTACCCAGCATCTAGGC
CTCC
CTCACCAGCCTCACCTTGTGGTGGCTTTTAAAGAGGATT
TAACTGTTTGTGGGGGAGGGAAGGGAAGAGAAGGAC
GGACGGGGTTTG
18 01
-GGGTATGAGGAC
CTTCTACCCCCGTGGTC
CCCTCCCCTCCCCCAGGCTAC
CACTCCTCCCCCCCCTCCCATGTCCCTTGTAAATAGAACCAGCCCAGCCGTATCCTCTTCCTGGCCCTTG
19
21-
GGTGTAAATAGATTGTTATAATTTTTTTC
TTAAAGAAAACGTCGATTCACACCATCCAACCT
GCCCTCCCCCTCAGCTGTACCCCCCTC
TTGTCCTCTGCTCCCAAGGCTTCCTCCCTCT
2
041-
CCCCATCCCAAGGAGGGGAGTAGGGAGAGCCCCTGGTGTCTTAGTTTCCACAGTAAGGTTTGCCTGTGTACAGACCTCCGTTCAATAAATTATTGGCATGAAA
AAAAAA
B
M
S
-2
1-
TTTT
TTCTTCGCGGGAGAACTTAATGCTGCATTTATTATTAACCTAGTACCCTAACATAAAACAAAAGGAAGAAAAGGACCAAGGAAGGAAAAGGTGAATCGAGAAGAGC
CAT
CAT
GTCG
G
R
P
R
T
T
S
F
A
E
S
C
K
P
V
Q
Q
P
S
A
F
G
S
M
K
V
S
R
D
K
D
G
S
K
V
T
T
V
V
A
-42
121
-GGGC
GACCGAGAACCACCTCCTTTGCGGAGAGCTGCAAGCCAGTGCAGCAGC
CTTCAGCTTTTGGTAGCATGAAAGTTAGCAGAGATAAAGATGGCAGCAAGGTAACCACAGT
GGTGGCA
T
P
G
Q
G
P
D
R
P
Q
E
V
S
Y
T
D
T
K
V
I
G
N
G
S
F
G
V
V
Y
Q
A
K
L
C
D
S
G
E
L
V
-82
2
41
-ACTC
CAGGACAGGGTC
CTGACAGGCCACAGGAAGTCAGTTACACAGACACTAAAGTCATTGGAAATGGGTCATTTGGTGTGGTATATCAAGCCAAACTTTGTGACTCAGGAGAACTGGTG
A
I
K
K
V
L
Q
D
K R
F
K N
R
E
L
Q
I
M
R
K
L
D
H
C
N
I
V
R
L
R
Y
F F
Y
S
S
G
E
K
-122
3
61
-GC
CATCAAGAAAGTTCTTCAGGACAAGCGATTTAAGAACCGAGAGCTCCAGATCATGAGAAAGCTAGATCACTGTAACATAGTCCGATTGCGGTATTTC
TTCTACTCGAGTGGCGAGAAG
K
D
E
V
Y
L
N
L
V
L
D
Y
V
P
E
T
V
Y
R
V
A
R
H
Y
S
R
A
K
Q
T
L
P
V
I
Y
V
K
L
Y
M
-162
4
81
-AAAGAT
GAGGTCTACC
TTAACCTGGTGCTGGACTATGT
TCCGGAAACAGTGTACAGAGTC
GCCAGACAC
TATAGTCGAGCCAAGCAGACACTCCCTGTGATCTAT
GTCAAGTT
GTATATG
Y
Q
L
F
R
S
L
A
Y
I
H
S
F
G
I
C
H
R D
I
K
P
Q
N
L
L
L
D
P
D
T
A
V
L
K
L
C
D
F
G
-202
6
0
1-TACCAGCTGTTCAGAAGTCTAGCCTATATCCATTCCTTTGGGATCTGCCATCGAGACATTAAACCACAGAACCTCTTGCTGGATCCTGATACAGCTGTATTAAAMCTCTGCGACTTTGGA
S
A
K
Q
L
V
R
G
E
P
N
V
S
Y
I
C
S
R
Y Y
R
A
P
E
L
I
F
G
A
T
D
Y
T
S
S
I
D
V
W
S
-242
7
21
-AGTGCAAAGCAGCTGGTCCGAGGAGAGCCCAATGTTTCATATATCTGTTCTCGGTACTACAGGGCACCAGAGCTGATCTTTGGAGCCACCGATTACACGTCTAGTATAGATATGTGGTCT
A
G
C
V
L
A
E
L
L
L
G
Q
P
I
F
P
G
D
S
G
V
D
Q
L
V
E
I
I
K
V
L
G
T
P
T
R
E
Q
I
R
-282
8
41
-GCAGGC
TGTGTGTTGGCTGAATTGTTGCTAGGACAACCAATATTTCCTGGGGACAGTGGTGTGGATCAGTTGGTGGAAATAATAAAGGTCCTAGGAACACCAACAAGGGAGCAAATTAGA
E
M
N
P
N
Y
T
E
F
K
F
P
Q
I
K
A
H
P
W
T
K
V
F
R
P
R
T
P
P
E
A
I
A
L
C
S
R
L
L
E
-322
9
61
-GAAATGAACCCAAATTATACAGAATTCAAATTCCCCCAAATCAAGGCACATCCTTGGACGAAGGTCTTTCGGCCCCGAACTCCACCAGAGGCAATCGCACTGTGTAGCCGTCTCC
TGGAG
Y
T
P
T
A
R
L
T
P L
E
A
C
A
H
S
F
F
D
E
L
R
D
P
N
V
K
L
P
N
G
R
D
T
P
A
L
F
N
F
-362
10
81-TACACGCCGACCGC
CCGGCTAACACCACTGGAAGCTTGTGCACATTCATTTTTTGATGAATTACGGGACCCAAATGTCAAACTACCAAATGGGCGAGACACACCTGCCCTCTTCAACTTT
T
T
O
E
L
S
S
N
P
P
L
A
T
I
L
I
P
P
H
A
R
I
0
A
A
A
S
P
P
A
N
A
T
A
A
S
D
T
N
A
-402
1201-ACCACTCAAGAACTGTCAAGTAACCCACCCCTGGCCACCATCCTTATCCCTCCTCACGCTCGGATTCAGGCAGCTGCTTCACCTCCTGCAAACGCCACAGCAGCCTCAGATACTAATGCT
G
D
R
G
Q
T
N
N
A A
S
A
S
A
S
N
S
T
-420
1321-GGAGACCGTGGACAGACCAATAACGCCGCTTCTGCATCAGCCTCCAACTCTACCTGAACAGCCCCAAGTAGCCAGCTGCGCAGGGAAGACCAGCACTTACTTGAGTGCCACTCAGCAACA
1441
-CTGGTCACGTTTGGAAAGAAAATTAAAAAAAAAA
2432