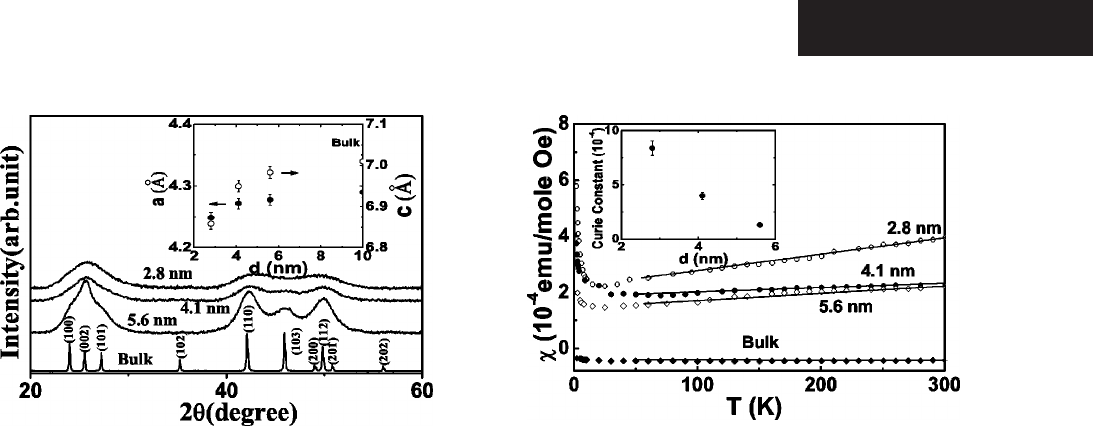
1-cm quartz cuvettes at room temperature as shown in Fig. 1.
The blueshift of the absorption edge with the decreasing d of
quantum dots is consistent with an earlier report.
12
X-ray
diffraction 共XRD兲 of the quantum dots 共carried out with a
3-KW Philips diffractometer equipped with an array detector
based on a real-time multiple strip兲 showed the wurtzite
structure of the bulk CdSe but with the expected line broad-
ening with decreasing d 共Fig. 2兲. In addition, there is a
shrinkage of the lattice constants 共the inset of Fig. 2兲, due to
size effect, somewhat similar to that reported in the
literature.
13
No additional lines due to any impurity phase
could be detected in the XRD spectra.
A calorimetric study was made in the range of 0.4 to 10
K, using a thermal-relaxation microcalorimeter in a
3
He
cryostat.
14
Each milligram-sized sample was prepared by
lightly pressing fine powders together. It was then attached
with thermal-conducting N grease to a sapphire disk, having
two deposited thin films serving as heater and thermometer,
respectively. The heat capacity of the sapphire disk and
grease were measured separately, and used as addenda cor-
rection in data analysis. The relative precision and the abso-
lute accuracy of the calorimeter were confirmed to be within
3% by measuring the copper standard. Magnetization mea-
surements were performed as a function of temperature using
the Quantum Design superconducting quantum interference
device 共SQUID兲 magnetometer in the range 2 to 300 K. The
magnetic susceptibility of straw and capsule were measured
separately and subtracted from the data.
III. RESULTS AND DISCUSSION
The temperature dependence of magnetic susceptibility
for CdSe QD with size d=2.8, 4.1, and 5.6 nm and bulk
CdSe is shown in Fig. 3. For the bulk CdSe,
is diamagnetic
and temperature independent with the magnitude
⬇−43⫻10
−6
emu/mole in good agreement with the earlier
results.
15
We report that for QD,
is positive and it has a
strong temperature dependence, especially below 30 K. Also
the magnitude of
is larger for the smaller particles at all
temperatures, showing the effect of size on magnetism.
In general for pure semiconductors,
=
l
+
f
+
i
, where
l
is the temperature-independent lattice contribution,
f
is
the free charge carrier 共electrons and holes兲 contribution and
i
is the contribution from bounded carriers and dangling
bonds. For bulk CdSe,
f
,
i
Ⰶ
l
, leading to magnetic sus-
ceptibility determined by
l
, which is usually negative
共Fig. 3兲.
15
Shaldin et al.
16
have shown that in II-IV semicon-
ductors, vacancies and interstitial can occur during the
growth. For QD, such defects will be more prevalent as com-
pared to bulk materials because of the increase in the relative
surface area. Specific magnetic clusters created by the donor-
acceptor pairs can exhibit paramagnetic behavior.
17
On the
surfaces of semiconductors, the free dangling bond bears an
electron spin by nature and can make semiconductor surfaces
magnetic. These phenomena are expected to be more signifi-
cant in QD.
18
With these considerations in mind, we suggest that the
low-temperature Curie tail in
is most likely due to surface
dangling bonds. These surface dangling bonds result from
decreased coordination of the surface atoms of the QD. We
have fitted the low-temperature data for T⬍ 30 K to the
modified Curie law:
=
o
+C/T, where
o
is temperature
independent contribution mainly from Pauli paramagnetism
of
f
mentioned above. The details will be discussed later.
The fits are excellent with C=1.33, 4.0, and 8.38 共in units of
10
−4
emu K/mol兲 for d=5.6, 4.1, and 2.8 nm, respectively
共inset to Fig. 3兲. This rapid increase in C with a decrease in
d is due to increase in surface/volume ratio as d decreases.
Note that C=N
2
/3k
B
where N is the number of dangling
bonds/mol, each with effective magnetic moment
and
k
B
is the Boltzmann constant. If we assume spin S=1/2
with each dangling bond, leading to
=1.73
B
, then
N=13.5⫻ 10
20
/mol for d=2.8 nm, thus yielding the concen-
tration of the dangling bonds ⬇2000 ppm. For d=4.1 and
5.6 nm, a similar calculation yields the concentration ⬇1000
and 300 ppm, respectively. It is noted that in amorphous Si
and Ge, low-temperature magnetic susceptibility studies
yielded similar concentration of spin density due to dangling
bonds.
19
The increase in
with increasing temperature above 30 K
seen for the QD in Fig. 3 is another interesting feature of our
FIG. 2. X-ray diffraction patterns for the bulk and d=2.8-, 4.1-,
and 5.6-nm quantum dots. Inset: The size dependence of lattice
constants of a and c axes.
FIG. 3. The magnetic susceptibility as function of temperature
for the bulk and d=2.8-, 4.1-, and 5.6-nm quantum dots; the lines
are for eye’s guide. Inset: The Curie constant vs d.
NEELESHWAR et al. PHYSICAL REVIEW B 71, 201307共R兲共2005兲
RAPID COMMUNICATIONS
201307-2