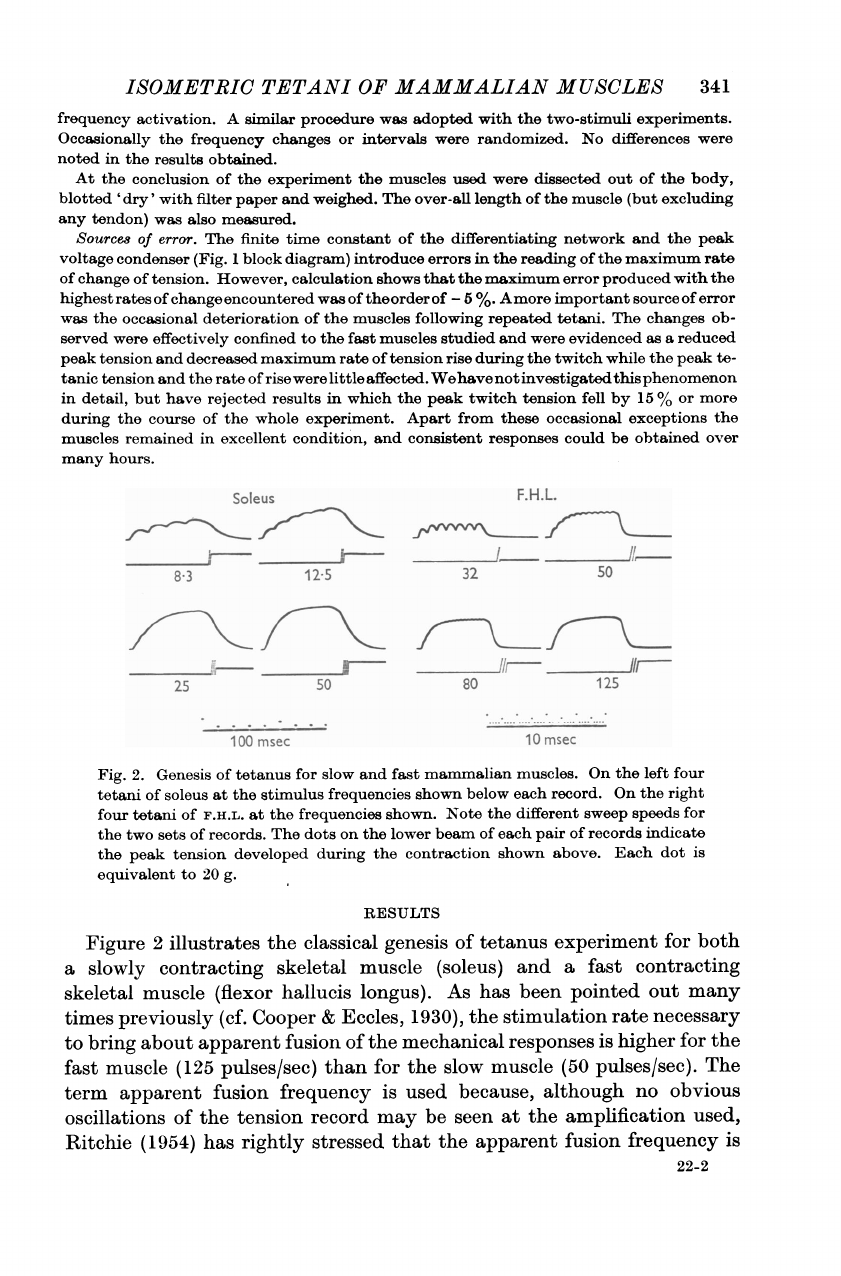
ISOMETRIC
TETANI
OF
MAMMALIAN
MUSCLES
341
frequency
activation.
A
similar
procedure
was
adopted
with
the
two-stimuli
experiments.
Occasionally
the
frequency
changes
or
intervals
were
randomized.
No
differences
were
noted
in
the
results
obtained.
At
the
conclusion
of
the
experiment
the
muscles
used
were
dissected
out
of
the
body,
blotted
'dry'
with
filter
paper
and
weighed.
The
over-all
length
of
the
muscle
(but
excluding
any
tendon)
was
also
measured.
Source8
of
error.
The
finite
time
constant
of
the
differentiating
network
and
the
peak
voltage
condenser
(Fig.
1
block
diagram)
introduce
errors
in
the
reading
of
the
maximum
rate
of
change
of
tension.
However,
calculation
shows
that
the
maxrimum
error
produced
with
the
highest
rates
of
changeencountered
was
of
theorderof
-5
%.
Amore
important
sourceof
error
was
the
occasional
deterioration
of
the
muscles
following
repeated
tetani.
The
changes
ob-
served
were
effectively
confined
to
the
fast
muscles
studied
and
were
evidenced
as
a
reduced
peak
tension
and
decreased
maximuim
rate
of
tension
rise
during
the
twitch
while
the
peak
te-
tanic
tension
and
the
rate
of
risewerelittleaffected.
Wehavenotinvestigatedthisphenomenon
in
detail,
but
have
rejected
results
in
which
the
peak
twitch
tension
fell
by
15
%
or
more
during
the
course
of
the
whole
experiment.
Apart
from
these
occasional
exceptions
the
muscles
remained
in
excellent
condition,
and
consistent
responses
could
be
obtained
over
many
hours.
Soleus
F.H.L
83
12-5
32
50
25
50
80
125
100
msec
10
msec
Fig.
2.
Genesis
of
tetanus
for
slow
and
fast
mammalian
muscles.
On
the
left
four
tetani
of
soleus
at
the
stimulus
frequencies
shown
below
each
record.
On
the
right
four
tetani
of
F.H.L.
at
the
frequencies
shown.
Note
the
different
sweep
speeds
for
the
two
sets
of
records.
The
dots
on
the
lower
beam
of
each
pair
of
records
indicate
the
peak
tension
developed
during
the
contraction
shown
above.
Each
dot
is
equivalent
to
20
g.
RESULTS
Figure
2
illustrates
the
classical
genesis
of
tetanus
experiment
for
both
a
slowly
contracting
skeletal
muscle
(soleus)
and
a
fast
contracting
skeletal
muscle
(flexor
hallucis
longus).
As
has
been
pointed
out
many
times
previously
(cf.
Cooper
&
Eccles,
1930),
the
stimulation
rate
necessary
to
bring
about
apparent
fusion
of
the
mechanical
responses
is
higher
for
the
fast
muscle
(125
pulses/sec)
than
for
the
slow
muscle
(50
pulses/sec).
The
term
apparent
fusion
frequency
is
used
because,
although
no
obvious
oscillations
of
the
tension
record
may
be
seen
at
the
amplification
used,
Ritchie
(1954)
has
rightly
stressed
that
the
apparent
fusion
frequency
is
22-2